Bacteria Make Sense
Talking BacteriaTo the eyes of a god, mankind must appear as a species of bacteria - Aleister Crowley by Marguerite Holloway Microbes seem to talk, listen and collaborate with one another - fodder for the truly paranoid... It is far too early in the morning, and Bonnie L Bassler is charging across the Princeton University campus, incandescent purple coat flying, brown curls bouncing, big laugh booming. She has come directly from the aerobics class she teaches every morning at 6:15 - "I get up at exactly 5:42, not a minute earlier, not a minute later," she says emphatically. She says most things with similar energy, and when the conversation turns to her work, she becomes, impossibly, even more dynamic. "I am not meant to be stopped in time," she laughs. "I am supposed to be a blur." The 41-year-old Bassler - a professor of molecular biology, winner of a 2002 MacArthur Foundation genius award, and occasional actress, dancer and singer - studies bacteria and how they communicate among their own kind and with other species. Quorum sensing, as this phenomenon is called, is a young science. Until recently, no one thought bacteria talked to one another, let alone in ways that changed their behaviour, and Bassler has been instrumental in the field's rapid ascension. She has figured out some of the dialects - the genetic and molecular mechanisms different species use - but is best known for identifying what might be a universal language all species share, something she has jokingly referred to as "bacterial Esperanto." As its moniker suggests, quorum sensing describes the ways in which bacteria determine how many of them there are in the vicinity. If enough are present (a quorum), they can get down to business or up to mischief. For instance, millions of bioluminescent bacteria might decide to emit light simultaneously so that their host, a squid, can glow - perhaps to distract predators and escape. Or salmonella bacteria might wait until their hordes have amassed before releasing a toxin to sicken their host; if the bacteria had acted as independent assassins rather than as an army, the immune system most likely would have wiped them out. Researchers have shown that bacteria also use quorum sensing to form the slimy biofilms that cover your teeth and eat through ship hulls and to regulate reproduction and the formation of spores. If it all holds up, the implications are enormous. Quorum sensing offers a way to think about evolution. Perhaps early bacteria communicated, then organised themselves according to different functions and, ultimately, into complex organisms. More practically, quorum sensing provides a strategy for medicine: muck up the communication system of dangerous bacteria, such as antibiotic-resistant enterococcus, and perhaps the bugs can't so effectively orchestrate their assault. As Bassler puts it, "You can either make them deaf or you can make them mute." The study of quorum sensing has its roots in the late 1960s. Two scientists - J Woodland Hastings and Kenneth H Nealson - discovered that a marine bacterium, Vibrio fischeri, produced light when its population reached a critical size. When fewer were present, the bacteria didn't bioluminesce. The two researchers speculated that the bacteria released a signal - something they called an autoinducer - that cried out, like Horton the elephant's dust speck in the Dr Seuss book, "We are here! We are here! We are here! We are here!" When the cacophony became loud enough, the assemblage glowed. In 1983 Michael R Silverman, then at the Agouron Institute in La Jolla, California, and a colleague identified the genes for V fischeri's autoinducer and its receptor. Bassler came to work with Silverman in 1990, after finishing her doctorate at Johns Hopkins University. She decided to focus on another glowing marine bacterium, V harveyi, to determine whether its signaling system was similar. She got to work making mutant bacteria - disabling a gene here, a gene there, to see if she could impair the one that triggered the bug to bioluminesce when it was in like company. "You turn off the lights in the room and just look for the ones that are dark when they should be bright or bright when they should be dark. It is genetics for morons," she quips. Bassler found the genes for V harveyi's autoinducer and its receptor. She also discovered something surprising. If she knocked out those two genes and put the altered V harveyi in mixed company - that is, around masses of different species of bacteria - it glowed. "So I knew there was a second system," Bassler remarks. Bacteria "don't have enough room in their genome to be stupid, so there had to be a separate purpose for this system." The foreign bacteria were emitting something that V harveyi responded to. Bassler called that something autoinducer two (AI-2). In 1994, as the field of quorum sensing was coming alive, Bassler moved to Princeton. Over time, she and others showed that quorum sensing initiates the release of toxins by bacteria such as V cholerae. And they found that every bacterium they tested has its own personal autoinducer, the one it uses to communicate with its own kind. Gram-negative bacteria such as Pseudomonas aeruginosa use different versions of AHL molecules (acylated homoserine lactones); gram-positive bacteria such as Staphylococcus aureus use peptides. But most bacteria Bassler looked at also used AI-2. By 1997 "we could see that all these bacteria made this molecule and that it was not just weird, crazy bacteria from the ocean," Bassler recalls. "So we got the idea that the bacteria must have a way of knowing self from other." For Bassler, the idea that different bacteria chat makes perfect sense. "There are 600 species of bacteria on your teeth every morning, and they are in exactly the same structure every single time: this guy is next to that one, is next to that one," she says. "It just seemed to us that you can't do that if the only thing you can detect is yourself. You have to know ‘other.'" Bassler and her students set out to purify and characterise AI-2. Finally, through the efforts of postdoctoral student Stephan Schauder and the crystallography of Frederick M Hughson and Xin Chen, they got it. AI-2 is an unusual package - a sugar with a boron sitting in the middle of it. "What is amazing about that molecule is that it is the first ever to have a biological function for boron. Ever!" Bassler exclaims. Now Bassler and her colleagues are trying to determine whether AI-2 is, indeed, one molecule that works alone as a signal and does not combine with other molecules to give rise to slightly different "languages." If it is the latter, no more Esperanto. "Her work has been truly superb," comments microbiologist Richard P Novick of New York University. "But there is argument about where [AI-2] comes from and why. And what role it plays in different systems is unclear." Some scientists are also concerned that aspects of quorum sensing - but not Bassler's findings - have been slightly overinterpreted. "Do bacteria want to communicate with each other, or is it just by accident?" asks Stephen C Winans, a microbiologist at Cornell University. "This idea has taken hold that these bacteria want to communicate with each other. It may be just too good to be true." Bassler's drive - her friend and former mentor Silverman describes her as "intensely motivated," "on a quest" and "just fierce" - suggests that she will hear bacteria's every last word. For the time being, she remains focused on understanding AI-2. "I want it all to be one thing, so I am sure that is wrong," she says. "I want it to be one thing because that is better if you want to make a drug, right?" Bassler is one of several quorum-sensing researchers working with companies to develop drugs. In 1999 she formed a company called Quorex with a former colleague from Agouron. Although her involvement is limited at the moment, she is hopeful that the start-up will find new antibacterials. "This was really considered fringe science," Bassler says. "Now it is this amazing field that didn't even exist 10 years ago." Marguerite Holloway is a contributing editor for Scientific American. Source: sciam.com February 2004 Microminds: the Minds of Microbesby Paul Pietsch The human cerebrum is the pride of the thinking world. On its convoluted hemispheres ride the star performers of the nervous system, the 14 billion temperamental neurons that, with their numberless satellites, form the cerebral cortex: 6 tiers of near liquid, delicate to the point of improbability, sculpted as personally as a face and, under a microscope, like a clear night sky at sea. The cerebral cortex is inseparable from the human condition, from what we are as a species and who we are as individuals. What lies in more humble reaches of thought? On the low end of the living spectrum are the bacteria. Headless, heartless, brainless, with a primitive cell for an entire body, one DNA molecule for a chromosome and a life span measured in minutes, some species just make it under the qualifying wire as organisms. The bacteria seem capable of little more than acting and reacting like the spring of a jack-in-the-box. But the denizens of our bowels and toilet bowls, the E coli and their kissing cousins, have become the subjects of the most rigourously controlled behavioural biological investigations yet conducted. And though they possess not a single neuron, their "thought processes" may have much in common with your thought processes and mine. A few years ago, I prefaced a lecture on memory with a brief description of bacterial behaviour, but soon yielded the floor to a person with a wide grin and a big cigar. "Please forgive my interruption. But I must ask if, in effect, you're saying that the little stinkers think?" I had to admit that I was. For deciding, choosing, judging, data processing and discriminating add up to thinking. Indeed, it is much easier to document certain types of thought in microbes than it is in human beings. Over a century ago, bacteriologists learned that microorganisms swim toward or away from substances such as chicken soup and mop-pail disinfectant. A German biologist named Wilhelm Pfeffer reported a remarkable observation in 1883. He filled capillary tubes with admixtures of repellents and attractants. With the correct relative proportions, bacteria would swim into a tiny tube after the attractant, even though the repellent by itself would have chased them scurrying in the opposite direction. What Pfeffer's bacteria did is akin to a person braving a buzzing beehive to get hold of some honey. Call it chemical attraction or behaviour or something else, Pfeffer's bacteria had to analyse and compare stimuli and then make a positive choice about what to do. They had to make a decision. Decisions? Bacteria? After all! Pfeffer's observations mouldered in the archives for 90 years. But in 1974, in Science magazine, an article by two microbial biochemists, Julius Adler and Wung- Wai Tso, appeared. Its title: "Decision- Making in Bacteria." Using strains of E coli whose pedigrees were known down to the gene, Adler and his colleagues demonstrated a great many factors in bacterial behaviour. They identified specific molecules that attract and repel the microbes, and found that an attractant isn't necessarily a food or a repellent a poison. These are genuine sensory stimuli - like the whiff of hot pastrami - the lure being independent of potential nutrition or heartburn. Another valuable discovery was the nature of the stimulation. Bacteria "perceive" not the absolute amount but the concentration gradient of stimuli - the increasing strength of a stimulus closer to its source. Adler and others also studied mutant E coil that cannot be enticed by the very same stimulus that a "normal" microbe finds irresistible. Since mutations mean altered genes, and altered genes mean altered proteins, Adler and others could compare normal and mutant E coli to discover which proteins were involved in sensory perception. It wasn't long before they had isolated proteins that act as the microbes' sensory receptors. Biochemist Daniel Koshland, of the University of California, Berkeley, calls these molecules the bacteria's "eyes and ears." In addition to the sensors, a dozen other proteins have been identified as the data processors - the thinking parts of the bacteria's molecular brain. Koshland, now a leader in the pursuit of microbial mentality, had initially been intrigued to find that E coli and their cousins could detect a gradient at all. The analytical data indicated that the cells were discriminating one part per 10,000; that's equivalent to one of us detecting the difference between a jar with 9,999 pennies and one with 10,000. With this minuscule bit of information, the cell would have to think: "Aha! The trail to the goodies is getting hotter in this direction." But how does it do it? In answering the question, Koshland's group made a monumental discovery: bacteria have a memory. Maybe the cell senses a gradient in space: it could feel a stronger pinch on its "head" than on its "tail." But an E coli's ends are only 0.000039 of an inch apart. And the microbes are less than half that wide. Not much space, Koshland thought. A swim across or around a drop of sweat for an E coli would equal a couple of laps across the English Channel for us. Instead, what if a microbe judged the gradient over time? What if the cell remembers the sensation of a moment ago and matches its present perception against its recollections. Koshland and his colleagues designed a test of the alternatives that seems ingenious to me. When E coli are not in a gradient, they tumble randomly. But when they sense graded amounts of attractant, they immediately check the tumbling and swim smoothly on a straight course. Now what would happen if bacteria were in a medium with an attractant mixed in? With no gradient they'd be somersaulting. What if somebody suddenly mixed in more attractant and its molecules diffused throughout the solution? If cells analyse head-to-tail, they'd keep on tumbling because there would be more attractant, but still no gradient. On the other hand, if the cells remember the previous concentration, adding more attractant should fool them into thinking they're in a gradient. And if they do have memory, then they will check the tumbling and begin to swim stably. Koshland tried the experiment, and, sure enough, mixing in more attractant tricked the bacteria; they began to swim smoothly. This demonstration of bacterial memory is one of the true gems of present-day research. Why? Look up mind in a dictionary, and you'll see memory at the top of the list of synonyms. Memory is to the function of a brain what heat is to a fire: the sine qua non, that without which a brain ceases to be a brain. There are the inevitable critics of microbial mind power. After all, even by the standards of microcircuits, bacteria are very small. How can all that memory fit into such a tiny space? A human brain fires hundreds of cells when deciding to advance or retreat. A simple neural network in a cat may involve 100,000 interconnections. Indeed, next to even the tiny brain of a salamander an entire bacterium seems like a speck of cosmic dust in a galaxy of stars. If nothing else, though, science teaches its followers that similarities can hide in unlikely places. And holograms - those strange 3- D images - may bear a clue to intelligence in both microbes and human beings. Holograms share some unique properties with brains. Two generations ago, Karl Lashley showed that memory cannot be culled from the brain and divided into discrete bits and pieces. Lashley taught rats to traverse mazes. The display of memory became dimmer and dimmer as he removed more and more cortex from the animals. Yet even animals with the smallest trace of a cortex could make a good stab at the maze. Certain kinds of holograms can be severely damaged, but the tiny intact parts can produce a whole, if sometimes faded, image. How can this be? Holograms store images by recording the relative positions or phase relations, of light waves. Phase is built into all cyclic events, from light waves to waves in the ocean. And just as a tsunami and a ripple on a pond can have the same profile, so, too, phase relationships can be recorded in an area that is very large or very small. If memory functions on the same principle as the hologram, then it could fit into a much tinier space than a human brain, a space much smaller than even an E coli. It's the pattern of interaction between waves that counts. And the same pattern can have different dimensions. You recognise the president's profile in a new photo just as well as if you had met him in person. Now let me connect phase to our own brains. Waves carry signals in the form of variations, one wave as compared with another. In an AM radio broadcast, the signal takes the form of modulations of amplitude - of variations in the size of the waves. But in an FM (frequency modulated) broadcast, the information is literally variations of phase. Frequency and phase are not identical, but both depend on time. And if frequency changes, phase automatically changes too. The impulse of a particular nerve cell cannot vary in amplitude. Instead, when communicating with other cells, the neuron varies the frequency of its impulses. Thus, phase is built into the physiology of the human brain. But phase is right on the surface of the little E coli. E coli bristle with hairlike flagella, so called because they resemble little whips. But they don't snap and crack like a whip, nor do they work back and forth like an oar or flipper. Instead, each flagellum rotates 360ยบ around a central axis and affects the surrounding medium much as a ship's propeller would. A flagellum is driven by a motor made of proteins. Situated at the base of the flagellum, the motor can speed up, slow down, stop and go into reverse. The driving force comes from streams of protons - naked hydrogens - stored near the motor and released in volleys by the chemical action of the sensory processing system. The flagellum's motor is much like that of, say, an electric mixer, but the mixer's is driven by electrons instead of protons. When an E coli is randomly tumbling through the medium, its flagella are extended to the sides and are spinning out of synchrony with one another. If the motions of the flagella were sounds, the tumbling bacterium would generate veritable cacaphony. But when the cell detects a gradient, something spectacular happens. The flagella begin to spin in sync, and they wind harmoniously together into a rotating tail. The prickly little tumbler metamorphoses into a creature resembling a sperm. The newly acquired tail, its components rhythmically cycling to a common beat, propels the tiny swimmer along a smooth, straight course. As components of a tail, the flagella collectively execute the sonic equivalent of a symphony of survival. How does it do it? On the sensory side, 30 kinds of chemical receptors and a dozen or so proteins that function as data processors lie at or near the inner surface of the cell's membrane. A receptor, activated by a stimulus, fastens itself onto a data processor. The processor, in turn signals two oppositely acting enzymes in the cell membrane. These enzymes chemically change the proteins near the flagella in the cell membrane by either adding or removing a small chemical unit called a methyl group. Each time the group is added, protons are released to run the flagellar motor. Microbial MemoryFor those in search of the microbe's memory, there is a very interesting fact about the addition and removal of the methyls. The enzyme systems that perform the two tasks operate at different rates. Those that put on the extra group are faster than those that strip it off. Inside the cells, there remains a microscopic chemical record - a memory - of events that occurred a few seconds in the past. In the cyclic changes of methyl there is a phase lag - and phase allows for hologramic memory. The memory in the methyl lag is of the short-term, or working, variety, the sort of information an organism must forget quickly as circumstances change. For you or me, it might be the recollection that one of us had put some water on to boil. For an E coli, it is the recollection of a recently sensed concentration. But the working memory contains a version of information encoded in permanent programs. And bacteria seem to have long-term memory and other abilities of "thoughtful" creatures as well. Koshland points out that when a bacterium shifts from random tumbling to smooth swimming, the cell is simplifying a complex problem. Somersaulting through three dimensions, its flagella cranking every which way, the bacterium would have a dickens of a time sensing, analysing and figuring out which direction to take. Simplifying complicated problems is one of the hallmarks of intelligent behaviour. Intelligence has other features, one of which is the capacity to learn. Certain molecules act as sensory stimuli for all cells of a particular strain and can trigger behaviour the very first time the cell encounters them. But that's only part of the story. Some molecules become stimuli only if present while the cell is maturing. If a young bacterium does not encounter these molecules at a tender age, it will never develop the mechanisms to perceive them. Thus, bacteria operate by learning as well as by instinct. I have taught neuroanatomy since 1955 and in all that time have yet to see two identical human brains. Yet under the microscope, E coli all look alike. Thus, I was stunned by another set of Koshland's observations. Personality QuirksDespite identical genes, in spite of the same environment, individual E coli acquire individual quirks and continue to display them for the rest of their lives. Bacteria exhibit rudimentary personalities. They exhibit traits that are determined by neither heredity nor environment but by chance. A zone of uncertainty - of individuality, if you will - surrounds the neighbourhood of intelligence in even the lowliest form. And when we start to talk of individuality, that brings a microbial mind very, very close to the realm of human experience. It's not a question of people and bacteria being the same thing. Nor does it seem likely that genetic engineers can exploit the phase lag in the bacteria's flagellar motor to manufacture human minds in the test tube. But the meek and the mighty perform similar tricks and display common principles. The utter simplicity of the microbial mind brings us nearer to the meaning of our own rub. References and Additional ReadingsAdler, J and Tso, W-W. 'Decision'-making in bacteria: chemotactic response of Escherichia coli to conflicting stimuli. Science 184: 1292 - 1294, 1974. Berg, Howard, C. Dynamic properties of flagellar motors. Nature 249: 78 - 79, 1974. Fridovich, Irwin. Bacterial chemotaxis. Britannica Book of the Year, 1983: 449 - 495. Koshland, Daniel E, Jr. A response regulator model in a simple sensory system. Science 196: 1055 - 1063, 1977. Koshland, Daniel E, Jr. Bacterial chemotaxis in relation to neurobiology. Annual Review of Neurosciences 3, edited by Cowan, W C et al, Annual Reviews, Incorporated, Palo Alto, 1980 pp. 43 - 75. Lewin, Roger. Is your brain really necessary? Science 210: 1232 - 1234, 1980. Silverman, Michael and Simon, Melvin. Flagellar rotation and the mechanism of bacterial motility. Nature 249: 73 - 74, 1974. Paul (Andrew) Pietsch, Phd Based on an article in the October, 1983 issue of Science Digest Source: indiana.edu The Creativity of MicrobesOur genes work in networks, much like circuits made of elements wired together in various ways. As genes are accidentally duplicated, mutated, and rewired, old networks can give rise to new ones. It's pretty clear our ancestors could have never become particularly complex if not for this sort of network evolution. As they acquired nerves, muscles, and other tissues, animals needed to organise more and more genes into new circuits. Single-celled microbes, such as bacteria, don't live without gene networks - far from it! In fact, in many ways bacteria are more adept at network engineering than humans. Evolution has engineered the networks of bacteria with many of the same tricks that produced our own. As one bacterium divides into two, all sorts of mistakes can creep into its duplicating DNA. As one generation inherits gene networks from its parents, the networks can slowly change. But bacteria can also do something else we virtually never do: they can swap genes. The genes may be carried by viruses that jump from one bacterial host to another; in other cases, bacteria slurp up DNA from dead microbes and insert it into their own genomes. In still other cases, genes can spontaneously slice themselves out of one genome and get inserted in the DNA of a distantly related species. The most famous example of this process is antibiotic resistance. One reason that resistant bacteria can spread so quickly in a hospital is that inheritance is not the only way these microbes can get hold of the genes that can fight off a drug. Every now and then, the genes get transferred from one species to another; the lucky bugs that receive them soon outcompete their cousins who lack the defense. Horizontal gene transfer, as it's known, may involve a single gene or an entire network of genes. And when two networks arrive in an alien genome, they can combine together into a bigger network that can do something entirely new. Horizontal gene transfer gives bacteria an extra dimension of creativity. Our penchant for pollution has given bacteria a new opportunity to flaunt this extra creativity. Over billions of years, they evolved the ability to eat just about any source of carbon on the planet. But in the past century we have created synthetic chemicals that bacteria have never faced before (or faced in only tiny amounts). In many cases, these chemicals kill off most of the bacteria that encounter them. Over the years, though, strains have emerged that can not only survive exposure to these pollutants but can even devour them. Scientists have unpacked the genomes of these hardy microbes to figure out how they evolved a solution so quickly. It turns out that microbes are swapping genes and gene networks, and then assembling them into networks that can handle the chemical at hand. Last year, for example, scientists looked at the bacteria that thrive in ground water near a Texas Air Force base polluted with fuel. One strain of bacteria there can break down chlorobenzene with a series of enzymes. This chlorobenzene-destroying network actually is the product of two smaller networks that can each be found in other bacteria strains in the same ground water. One turns chlorinated benzenes into another compound known as chlorocatechol. The other breaks chlorocatechol down into smaller molecules. Only in the strain studied by the scientists did these two networks come together to create an entirely new kind of metabolism. These bacteria show an evolutionary nimbleness we will never enjoy. But it may be possible to harness them to clean up the messes we make. For more information, see this fascinating survey in the March issue of Nature Review Genetics. Source: corante.com 2 March 2004 There are 4 pages in the "bacteria" series. Hitting either the "Back" or "Next" buttons below will take you to other pages of bacteria facts... For articles on bacteria, centrioles, chairs, nebulae, asteroids, robots, memory, chirality, pain, fractals, DNA, geology, strange facts, extra dimensions, spare parts,
discoveries, ageing and more click the "Up" button below to take you to the Table of Contents for this Science section. |
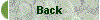
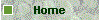
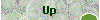
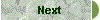